Biocementation is an environmentally friendly, cost-effective alternative to imported granular fills, concrete, costly hauling of materials or export to a landfill. In-service performance of soils, foundations, and other earthen structures and their required maintenance is highly dependent on methods of stabilisation, ranging from expensive mechanical stabilisation to chemical processes.
As such, many alternative materials originating from bio-based sources are being explored as potential stabilising additives to improve weak subgrade soils (i.e., dispersive soils, erodible and collapsible soil, and soft or expansive clays). In order to prevent geotechnical failures induced by water infiltration and/or erosion, some relevant alternatives include the use of bio-derived enzymes, microorganisms, and polymeric additives.
Bio-based Innovations in Geotechnical Engineering
For sustainable road infrastructure development, various microorganisms and other bio-based materials, including secondary metabolites, enzymatic materials, and polymeric materials, have been studied as viable substitutes for traditional chemical stabilisers (Ikeagwuani and Nwonu, 2019). Thus, increasing the adoption of these bio-based materials and technologies in the building industry requires a deeper understanding.
The use of bacteria in bio-based admixtures produced through industrial fermentation processes is gaining popularity because of a number of positive aspects, including their quick biosynthesis rates, high yields of desired products, quick growth on cheap culture media that is readily available in large quantities, openness to genetic manipulation, and lack of pathogenic traits (Pei et al., 2015). By the outcomes of the microbial treatment of soil, at least eight different types of biotechnological processes associated with construction can be categorized as shown in Figure 1 (Ivanov et al., 2015).
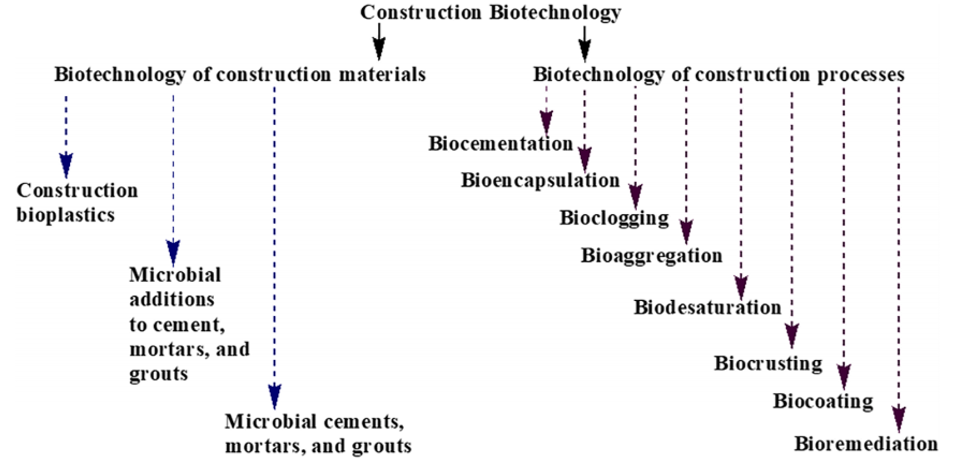
Of these, two biological processes (as far as the fundamental characteristics of research techniques) stand out;
(a) bioclogging, the production of pore-filling materials through biological means, to significantly reduce the hydraulic conductivity of soil or porous matrix and,
(b) biocementation, the generation of particle-binding materials through microbial processes in situ so that the shear strength of soil can be increased.
This is further reinforced by DeJong et al. (2010) stating that these methods allow a holistic view and meaningful characteristics in the area of soil stabilisation. The micro-organisms best suited for these techniques (i.e., soil bio-clogging, biocementation, and bio-aggregation) are facultative anaerobic and microaerophilic bacteria, although anaerobic fermenting bacteria have been suggested (Ivanov et al., 2015).
Microbial Biocementation
One of the most researched techniques is microbial-induced calcium carbonate precipitation (MICCP), also known as microbial biocement, which occurs when biological action increases the pH of soils to create supersaturated conditions (Oyediran and Ayeni, 2020).
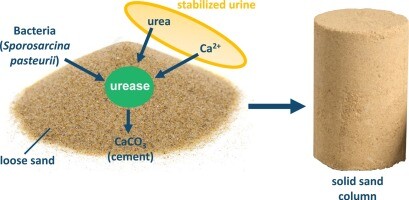
Recently, biocementation has gained attention as a practical method for soil improvement, notably because of its superior performance and environmental sustainability. For instance, an essential approach called microbially induced calcite precipitation (MICP) uses bacteria to create calcium carbonate precipitates and introduce “biocementation” between the sand grains (Cheng et al., 2013).
For instance, the alkalophilic soil bacteria Bacillus pasteuri uses the highly active urease enzyme to consume urea and break it down into carbon dioxide (CO2) and ammonia (NH3). In the presence of water, NH3 is changed into NH4+, while CO2 equilibrates with carbonic acid, carbonate, and bicarbonate ions in a pH-dependent manner. The alkaline environment and carbonate needed for the reaction with Ca2+ and precipitation of calcite (CaCO3) are provided by the rise in pH, as schematically depicted in Fig. 2.
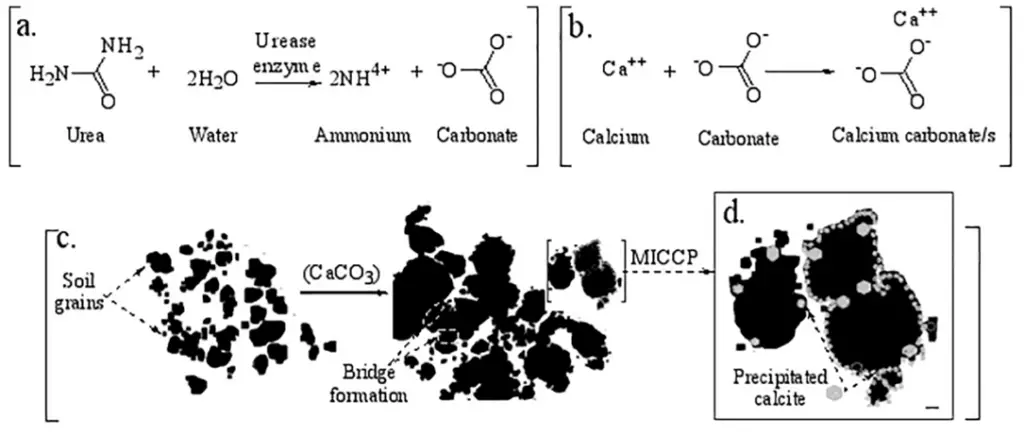
Another significant but understudied example of biocementation and bioengineered soil structures is termite mounds (anthills). Ecologists, entomologists, architects, and soil chemists are very interested in these above-ground mounds, particularly those made by the termites known as Macrotermitinae that grow fungi.
According to Kandasami et al (2016), a critical component for termite societies with millions of individual termites is the stability of termite mounds, which are bioengineered granular ensembles. Termites are skilled engineers, as shown by an experimental investigation on the mechanobiology of mounds and mound soil of the fungus-growing termite Odontotermes obesus (Rambur). The physical and mechanical characteristics of mound soil were notably different from those of the nearby or “control” soil. Yet, the clay mineralogy of the mound and surrounding soils was the same.
To build anthills, termites combine the finer soil fraction with their secretions to form boluses in the presence of water, thereby significantly altering the soil. In order to efficiently mould the soil, they control the amount of water close to the soil’s plastic limit when producing these boluses (Kandasami et al, 2016).
As a result of these processes, the strength of the soil can be increased tenfold as a result of the cementation caused by termites utilizing their excretions and/or secretions, which may not have been possible otherwise. The modification of the soil by termites reduces the likelihood of erosion and collapse of the mounds.
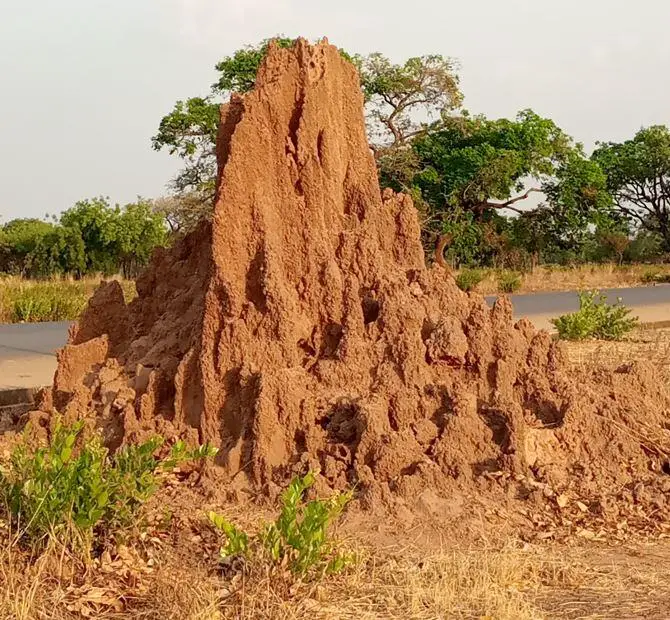
Studies have also shown that termites effectively bonded foreign objects, indicating that they have a variety of cementation skills. When compared to reconstituted soil, a slope stability analysis with intact mound soil showed a much higher safety factor for the mound.
Applications of Biocementation in Soil Improvement
According to Cheng et al (2013), the MICCP method is most efficient at a particle contact right as cementation begins, and it becomes less effective as cementation spreads outward around a particle contact. Due to the increased inter-particle interactions, reallocating the CaCO3 crystals to two contact places (connection points) as opposed to one would be more beneficial. As a function of the particle radius squared, the contact stress also decreases simultaneously. As a result, smaller particles have two complementary advantages: improved MICCP and reduced particle contact stresses.
Ng et al. (2012) used the B. megaterium MICCP procedure to evaluate shear strength, and they observed that the ratio of treated to untreated shear strength rose from 1.40 to 2.64. Van Paassen et al. (2010) reported a minimum of 300 kPa compressive strength. Similar results were published by Nafisi (2019), who observed that the soil strength varies between 210 kPa and 710 kPa depending on the type of sand and carbonate mass.
Van Paassen et al. (2010) also observed a 60% reduction in the permeability of treated soils at less than 100 kg/m3 CaCO3 precipitation; however, as solution concentration increased, calcite crystal clogged pore spaces and reduced permeability between 50 and 99% using 1 M cementation solution.
In triaxial testing, Cheng et al. (2013) reported that the mechanical behaviour of the bio-cemented sand increased the effective shear strength parameters (i.e., cohesion, angle of internal friction) with an increase in CaCO3 concentration at all saturation levels. By increasing the frictional angle at a lower saturation level, the precipitated crystals improved the cohesion of coarse sand. Under the same saturation level and similar CaCO3 content as fine sand, coarse sand showed a greater friction angle than fine sand.
Here is how the researchers addressed this: The primary consequences of tiny soil particles include:
MICCP method can be improved by;
(a) adding additional inter-particle contact points and
(b) lowering the stress per particle contact.
An exponential relationship between the UCS value and the CaCO3 content of the treated soils, according to van Paassen et al. (2010), demonstrates that even though the same amount of CaCO3 precipitated, the mechanical response can vary depending on the CaCO3 precipitation/crystals’ mechanism of action. Additionally, the process requires significant urease activity in the microorganisms, and the mode of action (MICCP) may be strain-dependent (van Paassen et al., 2010).
Several documented research works show several Bacillus spp. have the ability to precipitate calcium carbonate with structural properties, but a limited selection of micro-organisms highlights the triaxial and California Bearing Ratio (CBR) tests for potential road/pavement applications (Table 1).
Preferable microbes come from Bacillacae family | Key micro-organisms showing mechanical improvement of construction material |
B. flexus | Laboratory strength tests showed an increase in compressive (>40%), flexural (>30%) & split tensile (>10%) strength. X-ray powder diffraction (XRD) shows ureolytic properties of the organism (Roa et al., 2015). |
B. sphaericus | Experimental results indicate polyurethane immobilised bacteria-induced higher strength regain (60%) and lower water permeability coefficient (10 –11 m/s) (Wang et al., 2012, 2014) |
Sporosarcina pasteurii formerly known as B. pasteurii | Biocalcification mechanism showing potential construction applications such as dust control and strengthening of brick masonry (Sarda et al., 2009; Meyer et al., 2011). Triaxial tests indicate that the treated specimens exhibit a non-collapse strain softening shear behaviour, with a higher initial shear stiffness and ultimate shear capacity than untreated specimens. Samples treated showed a great increment in unsoaked and soaked CBR gained more strength when soaked for hours compared to unsoaked (Oyediran and Ayeni 2020). CBR obtained in a study showed poorer outcome from CBR index treated (0.6) compared to untreated samples (2.5). These investigations are of interest to improve soil stability, to build roads and paths, and to restore monuments (Morales et al., 2019). |
B. licheniformi | Calcium carbonate precipitation from the strain has the potential for sealing cement-based materials (Vahabi et al., 2015). |
B. subtilis | Strength performance of microbial concrete mechanism on the principle of calcite mineral precipitation by alkali-resistant spore-forming bacteria. Its response showed autonomous improvement in the self-healing process due to microcrack formation (Rao et al., 2017). |
According to Mujah et al (2016), the factors affecting the bio-cementation of soils are;
- Temperature
- pH level
- Urease activity/bacterial concentration
- Degree of saturation
- The concentration of cementation solution
Limitations of MICP for soil biocementation
The fact that MICP can only be applied to particular soil sizes is one of its key disadvantages. The approach is currently only effective for treating sands with particle sizes comparable to 0.5-3 mm, according to a review by Fragaszy et al (2011). Hence, expanding the use of MICP for improving and stabilising fine-grained soils like silt and clay would be a huge challenge.
Furthermore, some researchers have conducted field trials and upscaled studies to test the viability of MICP for in-situ bio-cementation deployment in construction. For instance, van Paassen (2009) conducted an experiment to treat 1-100 m3 of sand in the lab and observed that, after MICP treatment, the strength of bio-cemented sand was greatly increased; however, distinct spatial variability was observed. The most important factor that still needs further focus is treatment homogeneity or the homogeneous distribution of CaCO3 across the treated soil matrix from top to bottom.
Conclusion
The engineering, mechanical, and physical properties of biocemented soils can potentially be enhanced by MICP treatment. The intended uses of MICP include slope stabilization, settlement reduction, erosion management, soil self-healing, and prevention of liquefaction.
The benefits of MICP for soil improvement include cheaper costs compared to chemical grouting and other man-made materials, treatment dependability, and an overarching idea that encourages sustainability in tandem with future needs. Before the MICP method is directly applied in the field, further study should be concentrated on optimizing it at both the micro and macro levels.
Investigations must be done into specific issues such as medium flow and transport via heterogeneous media, treatment durability, mixing procedure permanence, and particle-level stratigraphic mapping. Further potential directions in MICP technology include the use of seawater as a salt alternative as well as self-healing in terms of pre- and post-shearing of biotreated soils after significant earthquakes and related aftershocks.
References
Cheng L, Cord-Ruwisch R, Shahin MA. (2013): Cementation of sand soil by microbially induced calcite precipitation at various degrees of saturation. Can Geotech J 2013;50:81–90. https://doi.org/10.1139/cgj-2012-0023
Dejong J. T, Mortensen BM, Martinez BC, Nelson DC. (2010): Bio-mediated soil improvement. Ecol Eng 2010;36:197–210. https://doi.org/10.1016/j.ecoleng.2008.12.029.
Fragaszy RJ, Santamarina JC, Amekudzi et al 2011. Sustainable development and energy geotechnology—potential roles for geotechnical engineering. KSCE J Civ Eng 15(4):611–621.
Ikeagwuani CC, Nwonu DC (2019): Emerging trends in expansive soil stabilisation: A review. J Rock Mech Geotech Eng 2019;11:423–40. https://doi.org/10.1016/j. jrmge.2018.08.013.
Ivanov V, Chu J, Stabnikov V. (2015): Basics of construction microbial biotechnology. In: Pacheco Torgal F, Labrincha JA, Diamanti MV, Yu CP, Lee HK, editors. Biotechnologies and biomimetics for civil engineering biotechnologies and biomimetics for civil engineering. Switzerland: Springer; 2015. p. 21–56.
Kandasami RK, Borges RM, and Murthy TG (2016): Effect of biocementation on the strength and stability of termite mounds. Environmental Geotechnics April 2016 Issue EG2 Pages 99–113 http://dx.doi.org/10.1680/jenge.15.00036
Meyer F, Bang S, Min S, Stetler L, Bang S. (2011): Microbiologically-induced soil stabilization: application of Sporosarcina pasteurii for fugitive dust control. In: Geo-frontiers 2011 Advances in geotechnical engineering; 2011. p. 4002–11.
Morales L, Garzon ´ E, Romero E, Sanchez-Soto PJ. (2019): Microbiological induced carbonate (CaCO3) precipitation using clay phyllites to replace chemical stabilizers (cement or lime). Appl Clay Sci 2019;174:15–28. https://doi.org/10.1016/j.clay.2019.03.018
Mujah D., Mohamed A. Shahin M.A, and Cheng L. (2016): State-of-the-Art Review of Biocementation by Microbially Induced Calcite Precipitation (MICP) for Soil Stabilization. GEOMICROBIOLOGY JOURNAL http://dx.doi.org/10.1080/01490451.2016.1225866
Ng WS, Lee ML, Hii SL. 2012. An overview of the factors affecting microbial-induced calcite precipitation and its potential application in soil improvement. World Acad Sci Eng Technol 6(2):683–689.
Oyediran IA, Ayeni OO. (2020): Comparative effect of microbial induced calcite precipitate, cement and rice husk ash on the geotechnical properties of soils. SN Appl Sci 2020;2: 1–12. https://doi.org/10.1007/s42452-020-2956-0
Pei R, Liu J, Wang S. (2015): Use of bacterial cell walls as a viscosity-modifying admixture of concrete. Cem Concr Compos 2015;55:186–95. https://doi.org/10.1016/j. cemconcomp.2014.08.007
Ramdas VM, Mandree P, Mgangira M, Mukaratirwa S, Lalloo R, Ramchuran S. (2020): Establishing miniaturised structural testing techniques to enable high-throughput screening of microorganisms and microbial components for unpaved road stabilisation application. J Adv Res 2020;21:151–9. https://doi.org/10.1016/j. jare.2019.11.002.
Rao MVS, Reddy VS, Sasikala C. (2017): Performance of microbial concrete developed using Bacillus subtilus JC3. J Insti Eng (India): Series A. 2017; 98(501–510). https://doi. org/10.1007/s40030-017-0227-x.
Roa R, Kumar U, Vokunnaya S, Paul P, Orestis I. (2015): Effect of Bacillus flexus in healing concrete structures. IJIRSET 2015;4:7273–80. https://doi.org/10.15680/ IJIRSET.2015.0408106
Sarda D, Choonia HS, Sarode D, Lele S. (2009): Biocalcification by bacillus pasteurii urease: A novel application. J Ind Microbiol Biotechnol 2009;36:1111–5. https://doi.org/ 10.1007/s10295-009-0581-4.
Vahabi A, Ramezanianpour AA, Sharafi H, Zahiri HS, Vali H, Noghabi KA. (2015): Calcium carbonate precipitation by strain Bacillus licheniformis AK01, newly isolated from loamy soil: A promising alternative for sealing cement-based materials. J Basic Microbiol 2015;55:105–11. https://doi.org/10.1002/jobm.201300560
Van Paassen LA, Ghose R, van der Linden TJ, van der Star WR, van Loosdrecht MC (2010):. Quantifying biomediated ground improvement by ureolysis: large-scale biogrout experiment. J Geotech Geoenviron 2010;136:1721–8.
van Paassen L. 2009. Biogrout: ground improvement by microbially induced carbonate precipitation. PhD Thesis, Delft University of Technology, Delft, Netherlands, p203.
Wang J, Soens H, Verstraete W, De Belie N. (2014): Self-healing concrete by use of microencapsulated bacterial spores. Cem Concr Res 2014;56:139–52. https://doi. org/10.1016/j.cemconres.2013.11.009.
Wang J, Van Tittelboom K, De Belie N, Verstraete W. (2012): Use of silica gel or polyurethane immobilized bacteria for self-healing concrete. Constr Build Mater 2012;26:532–40. https://doi.org/10.1016/j.conbuildmat.2011.06.054.
About article is very knowledgeable. Everyone should read it. Thanks for sharing